1. Introduction
One of the most common diseases in tomatoes (Solanum lycopersicum L.) is late blight, caused by the oomycete Phytophthora infestans (Mont.) de Bary. Foliar symptoms manifest as expanding dark lesions surrounded by water-soaked areas and, under favorable conditions, a halo of sporulation1. This pathogen can destroy the tomato crop in a matter of days due to its short life cycle and high sporulation rate2, causing production losses of up to 100%3.
Despite advances, fungicides remain essential in managing P. infestans due to the absence of resistant cultivars. The most effective strategy involves regular applications of both broad and narrow-spectrum fungicides, combining different defense mechanisms to robustly counter late blight and reduce chemical usage. This approach, while costly and potentially impactful on the environment, provides substantial benefits including reduced risk of pathogen resistance4.
The fungicides fosetyl-Al and fluoxastrobin are recommended for controlling late blight in potatoes and tomatoes. A study by Pirondi and others2 showed that fosetyl-Al maintains high efficacy in controlling the disease when applied after infection by P. infestans, which is significant for managing late blight in tomatoes. Also, Becktell and others5 assessed fungicides for controlling late blight in tomatoes and petunias under greenhouse conditions. They found that fosetyl-Al, as one of the treatments, suppressed late blight development. Furthermore, fluoxastrobin is advised for late blight control in tomatoes, with up to four applications per growing cycle, and should be alternated or mixed with a fungicide with a different mode of action6.
Plants employ defense mechanisms involving reactive oxygen species (ROS), such as superoxide anion (O2•-) and hydrogen peroxide (H2O2), inducing defense genes and enzyme production7. Enzymatic systems like superoxide dismutase (SOD) counteract O2•-, reducing the risk of OH• formation8. Peroxidase (POX) participates in redox reactions, influencing processes like lignification and protection against pathogens9. Phenylalanine ammonia lyase (PAL) is crucial for phenolic compound biosynthesis, contributing to defense10. Additionally, plant cells utilize non-enzymatic antioxidants like carotenoids and phenolic compounds, forming integral components of cell walls, providing resistance and induced defense11.
Several fungicides have a dual mode of action, that is, direct antifungal activity and activation of a certain level of induced resistance, such as fenpropimorph, metalaxyl, fosetyl-Al, copper hydroxide, DDCC, carpropamid, pyraclostrobin, and proquinazid, among others12. Additionally, it has been observed that certain fungicides induce non-specific defense reactions in plants, regardless of whether infection by a pathogen occurs 13)(14) . It has been observed that fosetyl-Al is metabolized into a phosphite ion, known to induce resistance when applied externally12, and stimulates the activity of antioxidant enzymes such as SOD and PAL in the presence of disease, inducing plant defense13. However, in the absence of disease, it has been shown to increase the activity of POX and SOD. Additionally, fluoxastrobin also induces SOD activity in plants14.
Analyzing how chemical fungicides affect defense responses in crops is crucial to understand how they can enhance plant resistance and improve resistance to foliar diseases15. Therefore, with the purpose of investigating how some fungicides affect defense mechanisms in tomato plants in a non-specific manner, and to understand whether the plant response is directed to the pathogen, the agrochemical or both, the objective of this work was to quantify the content of phenolic compounds and the activity of PAL, POX, and SOD in tomato plants with and without fungicides, in the absence and presence of P. infestans.
2. Materials and methods
An experiment was conducted under greenhouse conditions in Texcoco de Mora (State of Mexico, Mexico) for 12 weeks, from June to September 2016. Tomato hybrid EL CID F1 (HM Claus, Mexico) was sown in trays with a substrate consisting of 25% perlite and 75% peat moss. The trays were covered with polyethylene for two days. Subsequently, plants were watered daily until 25 days after sowing, and then transplanted.
The treatments consisted of foliar fungicide applications on both healthy and late blight (P. infestans)-infected tomato plants, including a control group for each. In total, there were six treatments, distributed in a completely randomized design with a factorial arrangement of 3×2 with 3 replications. The fungicide factor had three levels: Control, fosetyl-Al (Aliette WG®, Bayer CropScience, Mexico) at 1.25 g L-1, and fluoxastrobin (Vigold®, UPL, Mexico) at 2.5 mL L-1; while the plant factor had two levels: healthy plant (HP) and P. infestans-infected plant (IP). The fungicides and their doses were selected based on the study conducted by Serrano-Cervantes and others6, who found the induction of defense responses with these treatments in potatoes. Each experimental unit consisted of 20 tomato plants in 20 L pots with volcanic sand as a substrate, and they were fertilized daily with Steiner nutrient solution to 1.5 dS m-1.
To obtain the group of infected plants, inoculation was carried out with the JCh strain of P. infestans, previously isolated from cherry tomato leaves exhibiting late blight symptoms by Shakya and others16. This strain was multiplied in a liquid culture medium based on concentrated pea extract, sucrose, and distilled water in a ratio of 1:0.14:4. Inoculation was performed seven days after transplanting (dat) by applying a suspension of 4×104 spores mL-1.
The fungicides were applied at 20, 27, 34, 41, 48, and 55 dat using a manual pressure sprayer (Vivosun, United States) with a conical nozzle. To prevent the transmission of diseases from infected plants to healthy ones, and the drift of fungicides from one experimental unit to another, the experimental units were isolated with 200 μm polyethylene, covering the edges up to a height of 2.5 meters.
To determine the study variables, an initial leaf sample was taken at 18 dat, before the application of treatments, and subsequently at two and seven days after each treatment application, i.e., at 22, 27, 29, 34, 36, 41, 43, 48, 50, and 55 dat, totaling 11 samplings. The samples consisted of 40 g of leaves from the middle canopy of the plants for each treatment and replication. The leaves were cut at the base of the petiole using pruning shears. The plants from which samples were taken were excluded from subsequent samplings.
The severity of late blight was assessed in the 10 plants of each experimental unit using the severity scale proposed by Henfling17. Each plant was marked with a tape to be consistently evaluated over time. The assessment was conducted on the same days as the leaf sampling.
To determine enzymatic activity, acetone powder was prepared according to the methodology developed by Alia-Tejacal and others18. Starting with 40 g of leaflet, 100 mL of cold acetone was added, blended for 30 seconds, and vacuum-filtered. This process was repeated six times; the supernatant from the six extractions was mixed for subsequent analysis. The powder was allowed to dry at room temperature, the weight was recorded, and it was frozen for enzymatic analysis. The acetone extract was refrigerated for the determination of phenolic compounds.
The determination of PAL was carried out using the methodology described by Martínez-Téllez and Lafuente19, where 0.1 g of acetone powder was mixed with 5 mL of borate-sodium buffer (0.1 M, pH 8.8, 1% Polyvinylpyrrolidone) and 0.12% Mercaptoethanol. This mixture was homogenized at low temperature (T25 Ultra turrax, IKA, Wilmington, USA), filtered, and then transferred into centrifuge tubes. The mixture was centrifuged at 20,070 xg at 4 °C for 20 minutes using a Sorvall RC 6+ centrifuge (Thermo Scientific, Waltham, Massachusetts). Ammonium sulfate was added to the solution at a ratio of 0.46 g per mL and vigorously stirred. The tubes were then placed in an ice bath and shaken for 20 minutes at 15 °C (Max Q 4450, Thermo Scientific, Waltham, Massachusetts), followed by another centrifugation at 20,070 ×g and 4 °C for 20 minutes. For the phenylalanine ammonia-lyase (PAL) activity assay, two sets of tubes were used: the first set contained 4 mL of bidistilled water (pH 7.7) and 2 mL of extract; the second set contained 3.4 mL of bidistilled water (pH 7.7) and 2 mL of extract. Both sets were incubated at 39 °C in a water bath. After 10 minutes, 600 µl of L-phenylalanine were added to the second set, both were stirred, and readings were taken at 290 nm (Genesys 10 UV Scanning, Thermo Scientific, Waltham, Massachusetts). The samples were then incubated for 2 hours at 39 °C before taking another reading.
Peroxidase activity was measured using the method suggested by Flukley and Jen20. Beginning with 0.05 g of acetone powder in flat-bottom tubes, 5 mL of TRIS-HCl with PVP (0.1 M, pH 7.1, 1% Polyvinylpyrrolidone) were added. The solution was then homogenized at low temperature (T25 Ultra turrax, IKA, Wilmington, USA) for 30 seconds and centrifuged at 22,617 ×g at 4 °C for 30 minutes using a Sorvall RC 6+ centrifuge (Thermo Scientific, Waltham, Massachusetts). The enzymatic activity assay involved adding 2.5 mL of TRIS-HCl (0.1 M, pH 7.1), 0.1 mL of hydrogen peroxide (0.25%), 0.25 mL of guaiacol (0.1 M), and 0.15 mL of the sample. Measurements were taken at 30, 60, 90, and 120 seconds at a wavelength of 470 nm using a Genesys 10 UV Scanning spectrophotometer (Thermo Scientific, Waltham, Massachusetts).
The determination of superoxide dismutase was conducted using the method outlined by Beyer and Fridovich21. Initially, 0.05 g of acetone powder was placed in flat-bottom tubes, to which 5 mL of phosphate buffer (0.01M, pH 7.8) were added. This mixture was homogenized using a cold T25 Ultra turrax (IKA, Wilmington, USA) for 30 seconds. It was then centrifuged using a Sorvall RC 6+ (Thermo scientific, Waltham, Massachusetts) at 22,617 ×g and 4 °C for 30 minutes. In the absence of light, a mixture was prepared containing 81 mL of phosphate buffer + EDTA (0.01M, pH 7.8), 4.5 mL of L-methionine, 3 mL of nitroblue tetrazolium, and 2.25 mL of Triton X-100. To screw-cap tubes, 3 mL of this mixture and 500 µl of the sample were added. Three additional tubes were used as blanks, to which 3 mL of the mixture and 500 µl of phosphate buffer (0.1M, pH 7.8) were added. After vigorous shaking, 30 µl of riboflavin were added to each tube. The tubes were then exposed to fluorescent light for seven minutes, and the change in absorbance was measured at 560 nm.
The determination of phenolic compounds was carried out using the Folin-Ciocalteu methodology described by Waterman and Mole22. In this process, 150 µl of each extract was placed into flat-bottom tubes. Subsequently, 850 µl of distilled water was added and the solution was mixed. Next, 7 mL of distilled water and 500 µl of Folin-Ciocalteu reagent (2N) were incorporated. The mixture was allowed to stand for eight minutes before adding 1.5 mL of 20% sodium carbonate, followed by mixing and a two-hour rest in complete darkness. After the two-hour period, the samples were analyzed at a wavelength of 760 nm using a Genesys 10 UV Scanning spectrophotometer (Thermo Scientific in Waltham, Massachusetts).
Through the Shapiro-Wilk test, it was observed that the data from the variables do not follow a normal distribution (p < 0.05). Therefore, the non-parametric Kruskal-Wallis test (α = 0.05) was applied to analyze the main effects of each factor (Infection, Fungicide and Time) on the response variables. Subsequently, the Bonferroni post hoc (α = 0.05) was applied to detect which groups had significant differences. To understand the effect of variables over time, a methodology involving regression models was applied, based on sampling time and severity. The models were estimated using the method described by Volke23, which involves specifying an initial model with one or a few variables based on the graphical relationship between the response variables and the study factors. Additional variables were then incorporated into the model based on the graphical relationship between the residuals and the factors not yet included in the model that showed some response trend, until obtaining a model with a lower mean square error (MSE). The regression models were obtained using SAS 9.0 for Windows, and the graphs, in terms of sampling time and severity, were generated using the values estimated by the models.
3. Results
The Bonferroni post hoc test for late blight severity showed a significant effect of infection (p < 0.001), indicating a variation in blight severity between healthy and infected plants. The plants that were not inoculated with P. infestans maintained 0.0% severity of late blight throughout the experiment. However, there were no significant differences between the types of fungicides applied in infected plants (p = 0.990); fluoxastrobin reduced the severity of late blight to 16.7%, while fosetyl-Al reduced it to 35%, and the control showed 100% severity.
The application of fungicides had no effect on enzymatic activity or on the accumulation of PHE (Figure 1). Late blight severity also had no effect on the activity of PAL and SOD, which showed means of 0.019, and 92 U g-1 fresh weight, respectively (Figure 1a, Figure 1c). However, significant differences were observed in POX activity (p < 0.001), where healthy plants showed higher enzymatic activity (4793.8 U g-1 fresh weight) than infected plants (1858.1 U g-1 fresh weight) (Figure 1b). On the other hand, there was an effect on the accumulation of PHE in relation to severity and evaluation time. This response can be represented by the following model: PHE = 0.9499 + 0.06989 T - 0.000614 T2 + 0.0294 S - 0.00048 S2 (p = 0.0001; CV = 23.8%; CME = 0.378; R2 = 0.484).
Figure 2 illustrates the interaction between PHE accumulation, influenced by the study variables and determined by the regression model. The model reveals that the evaluation time affects PHE accumulation; as the plant matures, the level of these compounds decreases. Additionally, as late blight severity increases, the accumulation of phenols gradually declines. However, there is a significant relationship between time and severity; an increase in phenols is observed at 50 days after transplanting (dat), with blight severity ranging from 25 to 50%.
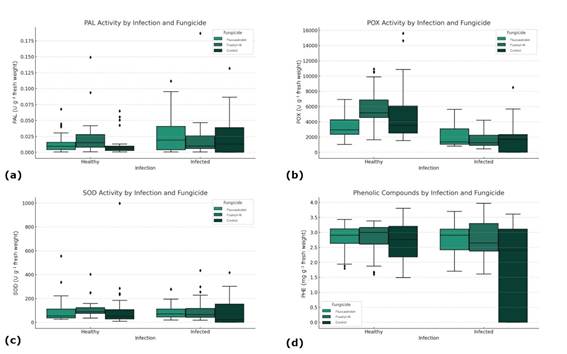
Figure 1: Influence of fungicide treatment and late blight infection status on biochemical activity in plants: (a) PAL activity by infection and fungicide; (b) POX activity by infection and fungicide; (c) SOD activity by infection and fungicide; (d) PHE concentration by infection and fungicide
4. Discussion
In our study, a complex response in the content of PHE was observed. Up to the first 27 dat and when the severity of late blight was low (< 1.5%), the levels of PHE in the plant were low. However, after 27 dat and/or as the late blight severity increased, a moderate increase in PHE levels was observed, likely as a plant response to the development of the disease24. Nonetheless, there comes a turning point where, after a long period (starting from 50 dat) or at high severity of late blight (> 18%), PHE production not only ceased to increase but may begin to decrease. This could be because the plant reaches a limit in its capacity to synthesize these compounds, or due to the damage caused by the disease. Similarly, Lozoya-Saldaña and others 10) found an increase in the accumulation of PHE in potato plants as the severity of late blight increased. Phytophthora infestans infection has an initial biotrophic phase that does not trigger cell death, synthesizing defense responses such as PHE through the salicylic acid pathway25. A decrease in the synthesis of defense compounds is observed during the necrotrophic phase, where the destruction of the host cell occurs26. In a similar study, Attia and others27 observed that eggplants (Solanum melongena L.) with symptoms of early blight (Alternaria solani) showed up to 77.21% more PHE at 60 days after planting, and up to 125.47% and 25.07% more activity of SOD and POX enzymes, respectively, compared to healthy plants. On the other hand, it has been observed that PHE production can vary depending on the plant genotype28. In this study, only a single hybrid was studied, so the responses of PHE content and enzyme activity in relation to infection and fungicides could vary with other materials.
Moreover, our results differ from those obtained by Serrano-Cervantes and others14, who found that, in the absence of disease, the application of foliar fosetyl-Al stimulates the activity of POX in potatoes, whereas, same to Di Marco and others29 observed that the activity of POX was lower in grapevine leaves infected by a fungal complex and treated with fosetyl-Al. Peroxidases are crucial for plant defense responses, catalyzing the oxidation of organic compounds using H₂O₂. However, severe, and continuous stress, such as that caused by pathogens, can lead to an overload of these enzymes, resulting in reduced activity30. Furthermore, peroxidase activity has been reported to decrease under stressful conditions like high temperatures31, which may also be linked to pathogenic stress. For instance, in the tomato-Botrytis cinerea pathosystem, a progressive inhibition of peroxidase activity has been observed in the advanced stages of infection, accompanying the development of disease symptoms. This could result from pathogen-induced senescence, thereby collapsing the protective mechanisms of peroxidases32.
In contrast to our results, Robledo-Esqueda and others13 found that the application of fosetyl-Al in potato plants infected by P. infestans stimulated the increase in PAL and SOD activity. Fosetyl-Al is primarily considered a fungicide due to its direct effect on target pathogens, but it can also induce defense mechanisms in plants33.
On the other hand, fluoxastrobin is a systemic fungicide belonging to the strobilurin family. It acts by inhibiting cellular respiration and is effective against oomycetes. However, evidence of its direct interaction with plant defense enzymes is limited. Serrano-Cervantes and others14 reported that a dose of 2.5 mL L-1 of fluoxastrobin stimulates the activity of SOD in non-pathogen-infected potato plants, which differs from the findings reported in this study. Other strobilurins, such as azoxystrobin, pyraclostrobin and kresoxim-methyl, have been reported to alleviate oxidative stress and stimulate the activity of antioxidant enzymes 34)(35) .
5. Conclusions
This study elucidates the complex relationship between fungicides, the severity of late blight, and certain tomato plants defense mechanisms. Given the experimental conditions and the plant material utilized, we conclude that fungicides may not trigger specific defense reactions in tomato plants, regardless of the presence or absence of disease. Remarkably, infection influences peroxidase activity, which is higher in healthy plants. Additionally, the trends observed in the accumulation of phenolic compounds underscore the importance of considering both the timing and severity of disease to fully understand plant responses to fungicide applications and late blight infection. Future studies should consider including a broader range of genotypes and isolates of P. infestans, a more extensive set of biochemical responses, and evaluations of the overexpression of genes related to plant defense.